In 1933, Fritz Zwicky, a Swiss American Astronomer, suggested there was something missing in the Universe, a missing mass.
While studying the Coma Cluster, Fritz discovered that the mass of all the stars in this cluster of galaxies contributed to an insufficient amount of mass.
It meant that the galaxies should have moved apart or there is some missing mass dwelling around, and fooling any detection.
What is the Coma Cluster?
The Coma Cluster is a large cluster of galaxies about 99Mpc or megaparsecs away from the Earth home to over 1,000 identified galaxies.
Today, we understand that all the visible, ordinary, or normal observable matter contributes only roughly ~5% of the actual entire mass of the Universe.
So where is the rest of it? Fritz wondered.
Discovery of Dark Matter
Astronomers speculate and attribute this missing mass to a combination of what they call now, dark matter and dark energy.
Dark matter is estimated to make up about 26.8% of the matter-energy composition of the universe while “ordinary” visible matter accounts for 4.9% and the rest 68.3 is attributed to dark energy[1].
If you consider the Universe analogous to a 1-liter capacity container filled with water, roughly 50ml of the water in there – about 10 teaspoonful – is the normal matter and the rest of it is dark matter and dark energy.
What is Dark Energy?
Dark energy is a hypothetical form of energy responsible for the accelerating expansion of the universe. Unlike matter, it has negative pressure, pushing apart the fabric of space-time.
It cannot be directly observed, but its existence is inferred from observations of cosmic microwave background radiation, supernovae, and large-scale structures in the universe.
Its exact nature is still unknown, and some theories suggest it could be a property of space itself or associated with a new fundamental particle.
Fritz Zwicky’s Observations – Missing Mass of the Galaxy Clusters
Fritz Zwicky’s work in 1933 was the very first one in the history of dark matter earlier termed “missing matter”.
Before his paper was published in 1933, Scientists believed that all the gravitational force in the universe was caused by the visible baryonic matter[2].
In his paper, he talked about an anomaly he and his colleague at Carnegie Mellon saw while observing the Coma Cluster[3].
He was studying the redshifts of various galaxy clusters from the experimental data published by Edwin Hubble and Milton Humason when he noticed a large scatter in the apparent velocities of eight different galaxies in the coma cluster whose differences were very large with respect to other clusters.
Single galaxies were moving too fast for the cluster to stay bound together as the observed mass of the cluster wasn’t sufficient.
This led them to believe that either such clusters were dissolving or something invisible existed, which Fritz Zwicky named Dunkle Materie or Dark Matter, the anomaly was, however, already observed by Hubble and Milton in 1931.
Who discovered Dark Matter?
The term dark matter was coined in 1933 by Fritz Zwicky of the California Institute of Technology to describe the missing mass inferred by observing the Coma Cluster.
Zwicky’s contribution was to apply the “Virial theorem” which wasn’t totally unheard of in the field to determine the mass of the cluster[4].
What is the Virial Theorem?
Virial Theorem relates the total kinetic energy of a self-gravitating body due to the motion of its constituent parts, T, to the gravitational potential energy, U, of the body.
$$2T+U=0$$
The general equation for the virial theorem is,
$$\langle T\rangle=-\frac{1}{2}\sum_{k=1}^{N} \langle F_{k} \cdot r_{k}\rangle$$
He estimated the total mass of the coma cluster to be the product of about 800 observed galaxies and their average masses which he took as 109 solar masses based on Hubble’s approximations.
Then in order to determine the potential energy of the system, he estimated its physical size as 106 light years and then calculated the average kinetic energy and then finally velocity dispersion.
He found that 800 galaxies of 109 solar masses in a sphere of 106 light years should exhibit a velocity dispersion of 80 km/s.
However, the observed average velocity dispersion along the line of sight was around 700 km/s.
From this, Zwicky concluded that the amount of dark matter present is much higher than the visible matter, his work relied on Hubble’s work on the relationship between redshift and distance.
In 1937, he wrote another paper in which he refined and extended his analysis of the Coma Cluster.
He used the Virial theorem this time as well, however, this time he estimated that about 1000 galaxies were contained within the radius of 2×106 light years in the coma and solved for its average mass.
From the observed velocity dispersion of 700 km/s, he obtained a lower limit of about 4.5×1013 solar mass on the mass of the cluster corresponding to the average mass of about 4.5×1013 solar mass per galaxy.
Then assuming that the average luminosity for cluster galaxies was about 8.5×107 times that of the Sun, the mass-to-light ratio came about to be 500[5].
Even after correcting some of the previous mistakes he made in accordance with the value of the Hubble constant, the coma cluster’s velocity dispersion still implied a very high mass-to-light ratio, this was the first-ever evidence of the existence of dark matter.
In 1936, Sinclair Smith estimated the mass of the Virgo cluster. His results were similar to that obtained by Zwicky.
Later, Smith was cited in Hubble’s The Realms of Nebulae where he stated that he considered the discrepancy between the masses of the galaxies inferred from the dynamics of clusters and those from the rotation of galaxies to be real and important.
He stated that this problem could be diminished by observing that the former were all likely in upper limits and the latter in lower limits.
However, Fritz Zwicky himself wasn’t fully convinced with his own argument[5].
Later, dark matter was forgotten for quite a while since no other Astronomer could find any more evidence regarding it until Vera Rubin’s pioneering work almost four decades later.
Vera Rubin’s Research – Flat Rotation Curves of Spiral Galaxies
It was in 1965 when two brilliant Astronomers namely Vera Rubin and Kent Ford met. Kent Ford’s lab was known to have the most sensitive spectroscope available at that time.
It had photomultipliers installed in it which allowed scientists to observe small regions of galaxies instead of simply entire objects.
Rubin and Ford started observing the nearest galaxy to the one we reside in, the Andromeda galaxy(M31), with the following goals:
- To observe the velocity field and determine the mass of M31
- To study the variation of line intensity field from region to region as a function of distance from the nucleus
- To relate the velocities and line strengths to the spiral structure of M31[6].
In 1970, they published their observations in a paper, the optical data they took extended to 110 arc min away from the galaxy’s center and was also compatible with the radio measurements previously taken by Morton Roberts in 1966[5].
It was around that time that researchers started to come out and explicitly agree that additional mass was indeed needed in the outer part of some galaxies based on the comparison of the rotation curves predicted from photometry and the measured ones.
Assuming that if luminosity was a sure factor of mass (meaning that most of the mass of a galaxy is concentrated at its center) then objects near the center should’ve moved faster than those towards the edge.
The speed of rotation is determined by the gravity caused by mass[7].
Since most of the mass in a galaxy is concentrated towards the center, the gravitational pull acting at the center should be much greater than its effects at the extremities.
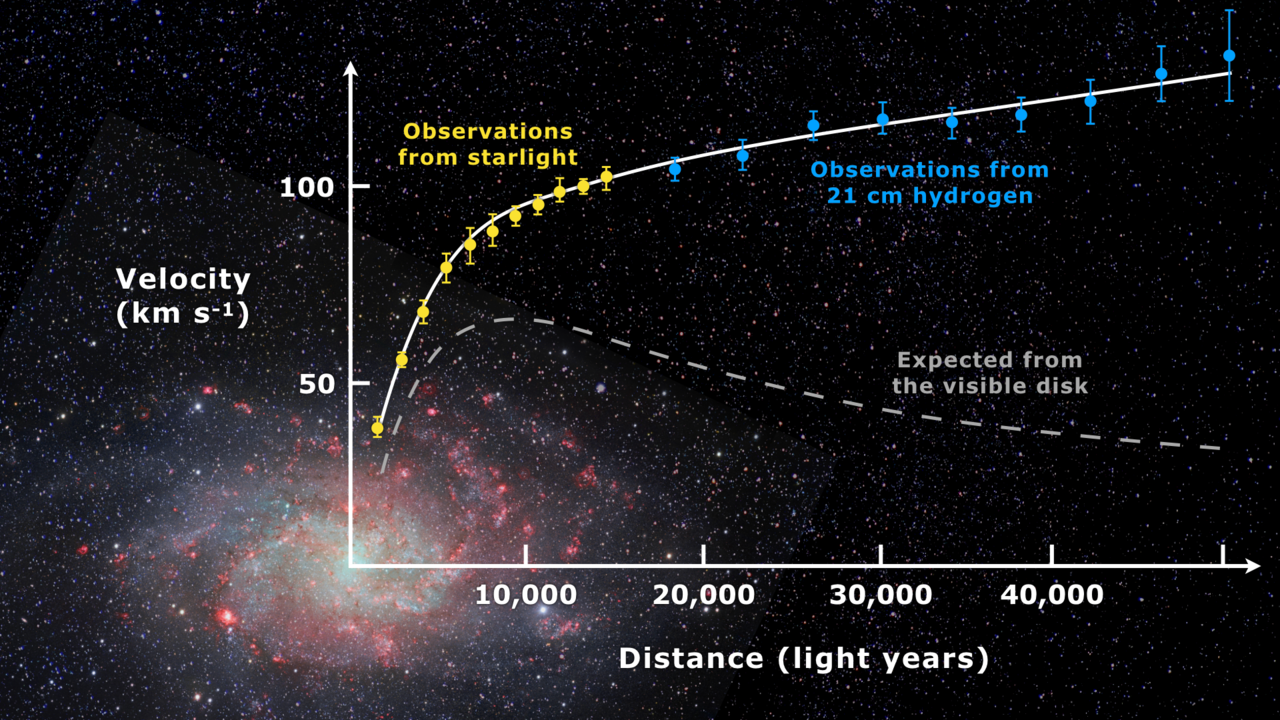
Instead, they observed that the rotational velocity of spiral galaxies either remains constant with an increase in the distance from the center or slightly rises, making the rotation curve flat.
Rubin concluded from this result that the fall in the amount of luminous mass, as one moves from the center to the edge, is balanced out by a non-luminous mass and this was when we finally got our second evidence that dark after indeed exists.
Evidence of the Existence of Dark Matter
In all, there are five pieces of evidence that prove the existence of dark matter, we have already partly discussed the anomaly in spiral galaxies, and the flat rotational curves, which were two of the five pieces of evidence.
Gravity Inferred Mass > Optically Inferred Mass
The gravitational force allows us to measure the mass of an object if we know the motion of some other object causing the gravitational field in the first place.
The scientists were observing the motion of a galaxy in a cluster of other thousands of galaxies, and what they observed proved to be peculiar given the knowledge one had at that time.
They saw that the gravitational inferred mass they’d calculated using the objects that could be “seen” was way lesser than that observed.
This was used by Fritz Zwicky in the 1930s to postulate that some invisible mass – dark matter – should exist in galaxy clusters.
Rotation Curve Anomaly of Spiral Galaxies
The circular velocity of the profile of the stars and gas in a galaxy as a function of their distance from the galactic center is called the rotation curve of a galaxy.
Basically, one would expect that mass is concentrated in the center of a galaxy going by the hypothesis that only visible baryonic matter exists so the rotation curve should be an increasing one.
However, in the 1970s Rubin observed that as one went from the center to the edge of the galaxy, the rotation curve was only slightly increasing or was totally flat.
This led her to believe that some unseen mass exists that is balancing out the fall of the seen mass.
Mass Bends Light by Virtue of Gravity
It is known that as a consequence of general relativity, light can be bent by the force of gravitation.
If a mass exists, it will definitely bend the light, the bending, however, depends on the magnitude of the mass.
Imagine there is a distant galaxy that we wish to observe and that there’s a galaxy cluster in between our home galaxy and the distant galaxy.
We already know that the galaxy cluster has more mass than the mass that is visible.
However, there’s another way to estimate the mass of the cluster, that is, by seeing how much it bends the light.
We observed through this method that the mass estimated by lensing is far larger than that inferred by the visible matter.
This piece of evidence had a lot of Scientists’ contributions, their time period spanning more than forty years from the early 1960s to the 2000s.
Density Fluctuations in Cosmic Microwave Background
We know that it is impossible for light to travel beyond opaque objects.
Let us imagine a scenario where an observer looks up at the sky and there are no opaque objects obscuring their vision.
If they look far enough, they’ll start seeing light from the universe immediately after the big bang.
The temperature of the young universe was so high that atoms could not stay bound together, leaving electrons and protons to just float around freely in an ionized plasma.
It’s impossible for the observer to look beyond this point.
No matter which direction they look in, there’s a particular distance after which the universe starts looking opaque.
Light from the youngest part of the universe that is observable arrives from when the universe became transparent when the universe cooled down enough.
This transition of the universe from being an ionized plasma to a transparent or neutral state is called recombination.
Right beyond the recombination is the oldest part of the universe acting like an opaque object, obviously whilst observing an opaque object, we not only see the things before it but also the surface.
Coming back to this surface, we know that the surface is so hot that it acts like a black body, and photons coming from this last scattering surface make up the Cosmic Microwave Background (CMB).
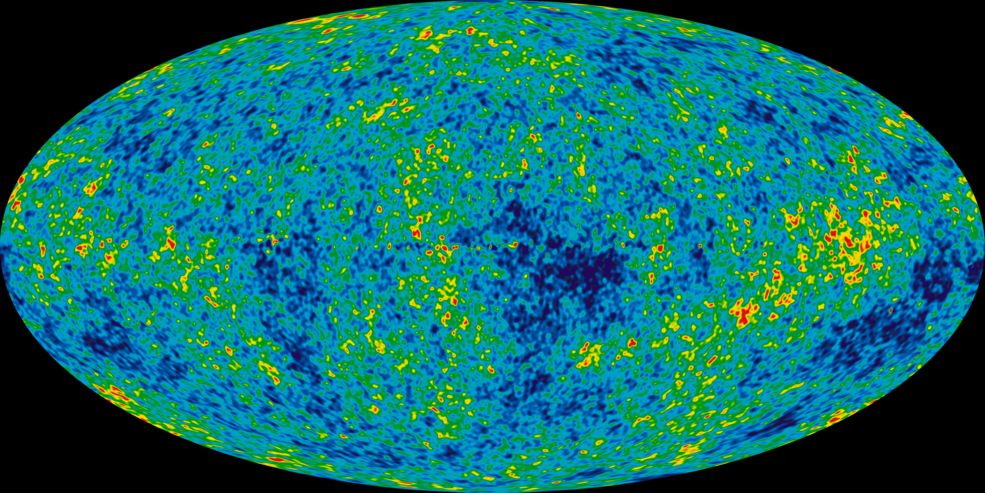
One may say that the CMB is pretty uniform, however, there are fluctuations in the density of the universe causing fluctuations in the temperature on this surface.
Looking at these fluctuations only, if there’s more mass at any point, the gravitational pull will cause it to collapse.
As the particles come closer together, they heat up and result in some sort of rise in the temperature, the heat radiation caused by the increase in temperature pushes out the particles.
This constant push and pull cause the surface to oscillate which in turn generates waves in the primordial universe and it is possible for us to calculate the amplitude and the wavelength of these waves.
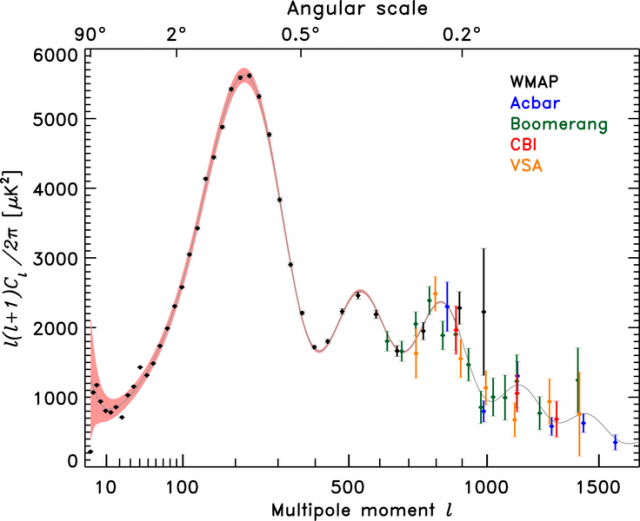
In the diagram above, the gray line represents the nature of the theoretical model while the points are the measurements taken by two satellites: WMAP and the Planck satellite.
As one can see, the nature of the theoretical model and that of the observed data totally line up with each other.
But catch this, it’s only after we add some mass, not just any mass though, but a mass that doesn’t electromagnetically interact with photons.
To get a clearer understanding let’s look back again.
So dark matter does contribute to the overall gravitational pull but visible matter is the only one that contributes to the radiation push.
The fluctuation we earlier talked about changed over time which depended on the gravitational pull of all matter.
However, the contribution of the dark matter to the gravitational pull was so high that it affected the density fluctuations of the normal matter.
These two different patterns did not quite line up with each other, so the dark matter did end up glorifying some changes in the normal matter while diminishing others.
As one can observe, the peaks in the diagram above get smaller with time but the second and the third peak are similar to each other.
This would not have been possible had the dark matter not existed since it increases the amplitude of the odd-numbered peaks while diminishing the even-numbered ones.
This is not only the fourth piece of evidence of the existence of dark matter but it also provides us with a key piece of information: dark matter does not participate in electromagnetic interactions[8].
Weird Collision of Galaxy Clusters
So far from all that evidence we’ve talked about till now, the most acceptable model we get is one that consists of dark matter: a matter with mass that doesn’t interact electromagnetically.
Let’s go back to 2005 when a group of scientists thought of a clever way to prove the existence of dark matter.
It’s very rare for galaxy clusters to collide but imagining that they do, its internal components behave differently.
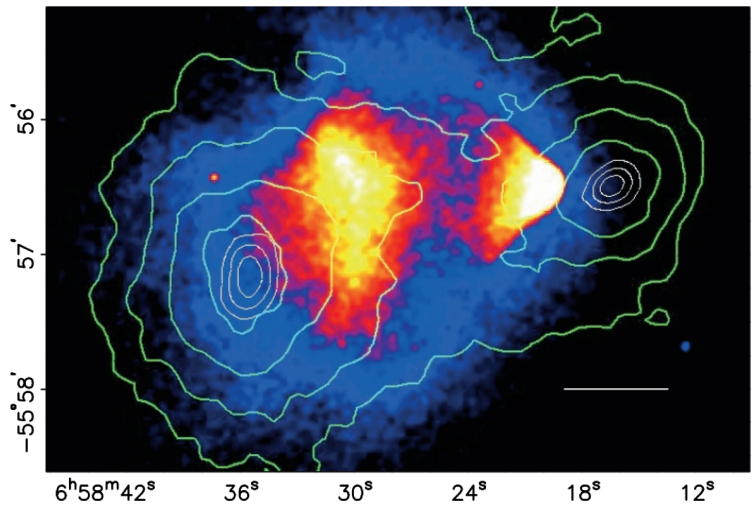
One expects the collision to be slow and heat up thus creating shocks and emitting X-rays.
Considering that there is no dark matter, all the gravitational lensing depends on the visible matter.
Instead, these gravitational lensing maps indicate that most of the mass is displaced from the visible matter.
The only two situations where this could happen without the existence of dark matter are if gravity is non-local or gravitating where the matter isn’t present.
But pre-merger clusters show us that gravity is local i.e. matter and gravity align with each other.
It is, of course, a given that colliding and non-colliding clusters should follow the same laws of gravity[9].
These 5 pieces of evidence support the mysterious existence of dark matter.
What is Dark Matter Made up of?
When we talk about dark matter, do we really have a specific particle in mind?
We’ve already established by valid pieces of evidence that dark matter does indeed exist.
We also know that, first, it interacts with visible baryonic matter so much that it disrupts our observations and second, it does not participate in electromagnetic interactions but is still stable for a long time.
This tells us that dark matter is not made up of known particles.
Why Baryonic or Normal Matter Can’t Be Dark Matter?
Baryonic matter makes up the visible part of the universe: planets, black holes, visible stars, etc following are the reasons why most of the dark matter couldn’t be made up of baryonic matter:
- The abundance of baryons is to be balanced out by the presence of other heavier elements. However, that is not the case; we know that baryons can only make up about 4-5% of the universe’s critical density.
- Gravitational microlensing in the Milky Way helped us observe that only a small fraction of dark matter can be in the form of dark, compact objects (Massive compact halo objects or MACHO).
- We talked about how in the cosmic microwave background, a large amount of mass interacts with photons only through gravity.
- Baryonic mass should be visible when backlit by stars.
Non-Baryonic Matter Maybe Dark Matter
After concluding that baryonic matter cannot make up most of the dark matter present, it is only logical for us to move on to non-baryonic matter.
Candidates for non-baryonic matter consist of hypothetical particles that might infer dark matter particles.
Neutrino as a Dark Matter Particle Candidate
It isn’t a big surprise that the first dark matter particle candidates were the three neutrinos (tau, electron, muon).
Neutrinos are stable and do not interact with electromagnetic or strong forces, the two most important features any dark matter particle must have.
In 1966, Zeldovich and Gershtein published a paper in which they considered the production of neutrinos shortly after the Big Bang.
With the cosmic background being discovered in 1965, they used this knowledge to predict how many electron and Muon Neutrinos would have existed in thermal equilibrium in the early universe and at what temperature those particles would have ceased to efficiently self-annihilate, leading to a population of neutrinos that survived as an aftermath of it.
Considering how the density of those neutrinos would impact the expansion history of the Universe, and comparing that to existing estimates of the Hubble constant and the age of the oldest observed stars, Zeldovich and Gershtein concluded that the masses of the electron and muon neutrinos must each be less than approximately 400 eV; if they had been heavier, the neutrinos would have unacceptably slowed, or even reversed, the rate of cosmic expansion.
For the muon neutrino, this result represented an improvement of 3 orders of magnitude over the previously existing upper limits[10].
They also showed that a neutrino species with a mass equal to or greater than a few tens of eV, although, they did not specify anything about them accounting for any of the missing mass.
After this work, a lot of other papers were published supporting it and researchers began to believe that weakly interacting particles may have had an observable impact on the evolution of the universe and were abundant.
In 1980, a few researchers studying tritium beta decay were able to measure the mass of the electron antineutrino(~30 eV).
With this mass, they were expected to have a large impact on cosmology.
Around the middle 1980s, numerical simulations were formulated, this allowed scientists to observe to see how dark matter evolves under the force of gravity in an expanding universe and to differentiate between particle candidates to some extent.
The main characteristic that these numerical simulations probed into was whether they were hot (referring to the relativistic matter) or cold (non-relativistic).
These simulations quickly proved that hot and cold matters lead to very different patterns of the large-scale structure.
It quickly became clear that hot dark matter could not account for most of the dark matter in the universe.
After that, it started to become more widely accepted that neutrinos did not make up most of the dark matter present.
Scientists also started to believe that at least one currently unknown particle must exist that makes up this non-visible mass.
Even though it was concluded that the existing neutrinos in the standard model could not make up dark matter due to it being far too hot and light, the possibility of currently undiscovered neutrino-like particles that fit into our model could not be ruled out.
In 1994, Dodelson and Widrow described a model where dark matter was in the form of sterile neutrinos which, unlike the neutrinos of the standard model, did not experience the electroweak force[10].
This was later proved to be wrong but its variants still remain one of the viable particle candidates.
Supersymmetric Particles Might Be Dark Matter
As early as the 1970s, Physicists began considering the existence of supersymmetry in nature which was basically spacetime symmetry relating Bosons to Fermions.
All it requires is that for every fermion, a boson with the same quantum numbers must exist and vice versa.
Therefore, it predicts the existence of several new electrically neutral and non-strongly interacting particles such as the superparticles of the Higgs boson, Neutrinos, Photons, etc.
Piet Hut’s paper in 1977 was the first ever to talk about graviton’s superparticle gravitino.
A 1982 paper by Heinz Pagels and Joel Primark, explicitly proposed gravitino being the solution to the missing mass problem.
However, at that time, supersymmetry itself did not have any concrete theoretical models.
In 1981, the Minimal Supersymmetric Standard Model (MSSM) was proposed by Savas Dimopoulos and Howard Georgi.
This model, in particular, talked about the Superpartners of the Photon, the Z boson, and two neutral Scalar Higgs bosons which later came to be known as Neutralinos.
To date, neutralinos are the single most researched particle candidate for dark matter.
The only problem one has faced in this theory yet is that the lightest Neutralino would require something to stabilize it.
Axions Maybe Good Dark Matter Particle Candidates
This is probably the second most studied particle candidate.
To understand axion, we must jump fields and understand the strong charge-parity problems faced in the Quantum Chromodynamics (QCD) theory which is otherwise very successful.
Looking at the QCD lagrangian,
$$\mathcal{L}_{QCD} \supset \bar\Theta\ \frac{g^{2}}{32\pi^{2}}G^{\alpha\mu\nu} \tilde G_{\alpha\mu\nu}$$
Where $G^{\alpha\mu\nu}$ is the gluon field strength tensor and $\bar\Theta$ is a quantity closely related to the phase of the QCD vacuum.
One would expect this quantity to be close to being of the order of one, this would however, introduce a big charge-parity violation causing the electric dipole moment of the neutron to be ~1010 times larger than the observed upper bound limits.
For it to be consistent with the experimental data, $\bar\Theta$ should be smaller than ~10-10.
The leading solution to this problem was introduced by Peccei and Quinn in 1977 where they showed that by introducing a new global U(1) symmetry that if spontaneously broken, could drive $\bar\Theta$ towards being close to zero.
In 1978, two scientists, Wilzeck and Weinberg, independently pointed out that this broken symmetry also indicates the existence of a Goldstone Boson called an Axion.
The axion acquires a small mass as a result of the U(1) symmetry’s chiral anomaly, on the order of $m_{\alpha}\sim \lambda^{2}_{QCD}/f_{PQ}$, fPQ where is the order at which the symmetry is broken and $\lambda_{QCD} \sim 200MeV$ is the scale of QCD[10].
However, later a lot of constraints were placed on the axion after some further observations. To dodge these restrictions, they had to be much lighter and more weakly interacting than as assumed before.
The Axions produced in the primordial universe were also stable on the cosmological scale so they must have had a decent amount of impact in its evolution and if they existed in good numbers, they could’ve constituted dark matter.
Classification of Dark Matter Based on Theoretical Models
As we’ve established before, dark matter isn’t thought to be made of just one particle.
In the 1980s, scientists started thinking that it was about time to classify dark matter into subgroups to help them study it better.
The particles were classified into three types namely, cold, warm, and hot, based on how fast they would’ve traveled in the early universe, since speed depends on the mass and also, on the temperature of their surroundings at the time of their formation.
Cold Dark Matter (CDM)
The slowest dark matter particles, namely, the cold dark matter particles seem to have taken part in building the structures, ergo, they must exist.
It is assumed to have existed since the beginning of the universe, there are no currently known cold dark matter particles yet.
The particle candidates for cold dark matter are: Weakly Interacting Massive Particles (WIMPs) and MACHOs[11].
Warm Dark Matter (WDM)
Warm dark matter particles are assumed to have properties that fall into the middle of the spectrum between hot and cold dark matter.
The most common warm dark matter particles are Gravitinos, Sterile Neutrinos, and Non-Thermally Produced WIMPs.
Hot Dark Matter (HDM)
Hot dark matter particles, traveling with a speed close to that of light, are supposed to be the fastest-moving dark particles to exist.
Their contribution to the universe would have been to smooth out any density fluctuations in the already-formed structures.
This is the reason why they are not considered to make up a large fraction of the dark matter population.
Neutrino is thought to be the foremost HDM particle candidate.
The density of neutrinos in the early universe was high enough to make up most of the matter density.
This would mean that density fluctuations could only have appeared when they would’ve slowed down enough[12].
The only particle candidate that we’ve previously talked about that has not been classified under any of the categories is the Axions. Axions are both light and extremely cold making them different from all the other particles which show either CDM, HDM, or WDM properties.
Most of the current theoretical models believe that a mixture of CDM and HDM make up the dark matter population.
However, a lot of theorists also believe that CDM could’ve been the only one as well[13].
So What’s Up With the Invisible Glue?
The pursuit of discovering the origins of our Universe has always been never ending and according to our current understanding, dark matter has played a key role in its formation.
To date, we have about five solid pieces of evidence that conclude that dark matter should exist according to relativistic mechanics.
- All the things we know about dark matter as of now are based on the theoretical models and observations of phenomena that differ from the expected result, we are yet to actually detect the particles.
- Dark matter particles do not interact with other particles or with themselves electromagnetically; they only interact through gravity.
- Most of the dark matter should be made up of Cold dark matter particles.
- Dark matter must make up a large population in the universe, much greater than the population of visible baryonic matter based on the perturbations observed.
- Its gravitational effects are mostly found in places where normal matter does not exist.
However, a lot of our questions remain unanswered, such as:
- Will we ever be able to detect dark matter directly?
- Is it eternally stable or will it decay away at some point in time?
- Is dark matter a mixture of particles or just one particle and what are they?
- Did dark matter always exist in the universe or was it made up later at some point?
What started as a cosmological problem has now become an interplay of particle physics and astrophysics, two of the most interesting fields to have existed in physics.
After over nine decades of research in the field, dark matter still remains a conundrum and many would agree that that is what makes it beautiful.
Recommendations
‘History of the Standard Model in Particle Physics‘, Evincism
‘Largest Map of the Universe – Dark Energy Survey‘, Evincism
‘Bizarre Large Scale Structure of the Universe‘, Evincism
References
- NASA, ‘Planck Mission Brings Universe Into Sharp Focus‘, 21 March 2013, “The new estimate of dark matter content in the universe is 26.8 percent, up from 24 percent, while dark energy falls to 68.3 percent, down from 71.4 percent. The normal matter now is 4.9 percent, up from 4.6 percent.”, https://www.nasa.gov/mission_pages/planck/news/planck20130321.html[↩]
- Arthur Miller, ‘A Short History of the Missing Mass and Dark Energy Paradigms’, Astronomical Society of the Pacific Conference Series, 2001, https://adsabs.harvard.edu/full/2001ASPC..252…75V[↩]
- Jaco de Swart, ‘Deciphering dark matter: the remarkable life of Fritz Zwicky’, Nature, 03 November 2019, https://www.nature.com/articles/d41586-019-02603-7[↩]
- Fritz Zwicky, ‘Republication of: The Redshift of Extragalactic Nebulae’, General Relativity and Gravitation, 18 November 2008, https://doi.org/10.1007/s10714-008-0707-4[↩]
- Gianfranco Bertone and Dan Hooper, ‘History of dark matter’, American Physical Society, 15 October 2018, https://journals.aps.org/rmp/abstract/10.1103/RevModPhys.90.045002[↩][↩][↩]
- Vera C. Rubin, ‘Dark Matter in Spiral Galaxies’, Scientific American, June 1983, https://www.jstor.org/stable/24968923[↩]
- Sara Scoles, ‘How Vera Rubin Confirmed Dark Matter’, Astronomy.com, 04 October 2016, https://astronomy.com/news/2016/10/vera-rubin[↩]
- Erik Anson, ‘What does the cosmic microwave background show us about dark matter? How?’ “In the early years after the big bang…”, Quora, 2019[↩]
- Ethan Siegel, ‘This is how galaxy cluster collisions prove the existence of the dark matter’, Forbes, 20 January 2020, https://www.forbes.com/sites/startswithabang/2020/01/20/this-is-how-galaxy-cluster-collisions-prove-the-existence-of-dark-matter/?sh=5e4d9b4a5b23[↩]
- Gianfranco Bertone and Dan Hooper, ‘History of dark matter’, American Physical Society, 15 October 2018, https://journals.aps.org/rmp/abstract/10.1103/RevModPhys.90.045002[↩][↩][↩]
- John P. Millis. ‘Cold dark matter’, ThoughtCo., 10 January 2020, https://www.thoughtco.com/cold-dark-matter-3072275[↩]
- Martin White, ‘Hot dark matter’, Berkeley, https://w.astro.berkeley.edu/~mwhite/darkmatter/hdm.html[↩]
- Glennda Chui, ‘Is dark matter cold, warm or hot?’, Symmetry Magazine, 10 December 2021, https://www.symmetrymagazine.org/article/is-dark-matter-cold-warm-or-hot[↩]